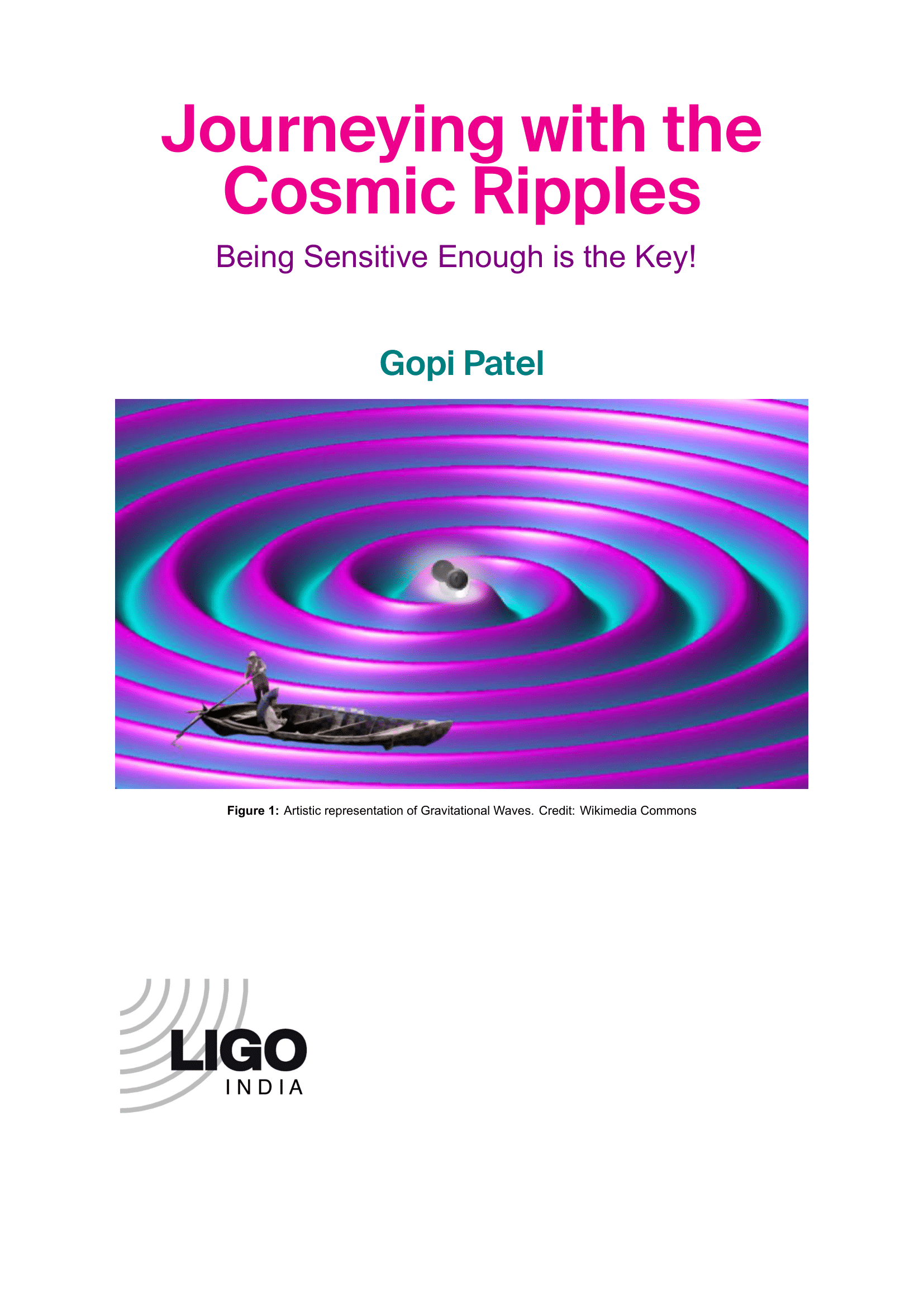
LIGO running to catch the ripples
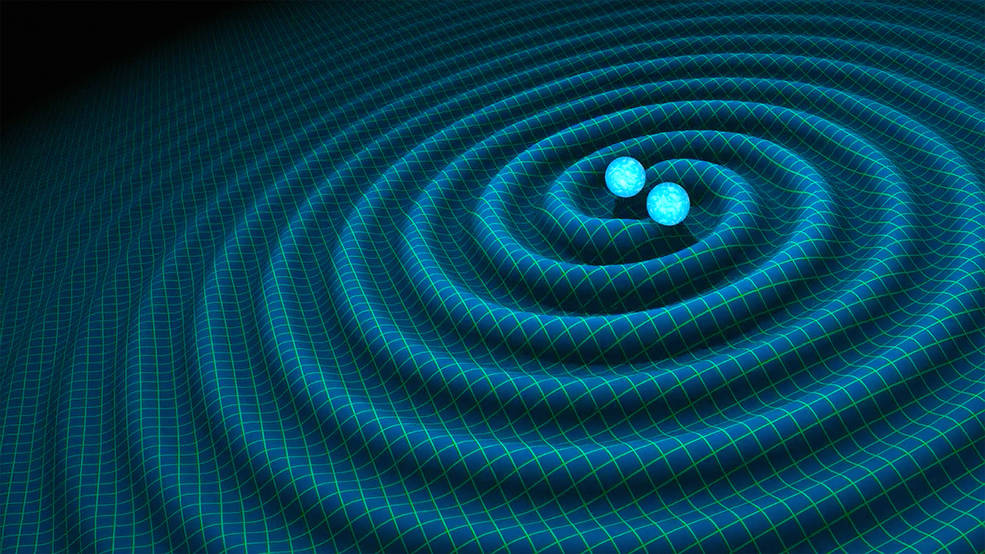
Figure 1: An artist’s impression of Gravitational-Waves generated by binary neutron stars. Credit: R. Hurt/Caltech
It has been seven years since we developed a sixth sense towards the Universe and detected the first Gravitational Wave signal - GW150914, which unfurled new vistas to survey and learn about our Universe. And, in March 2020, the Advanced LIGO and Advanced Virgo detectors completed their third observational run for Gravitational-Waves, detecting 90 compact binary coalescence candidate events, amongst which at least 50% are of astrophysical origin.
Gravitational-Waves are the ripples in the curvature of space-time generated by the violent activity of accelerated masses, like when neutron stars or black holes orbit each other. The disruption caused in space-time propagates at the speed of light outwards from its source. Even though the Gravitational-Waves originate from extremely energetic processes, by the time the waves reach Earth, they are thousands of billions of times weaker, making them particularly challenging to detect. Therefore, the LASER Interferometer Gravitational-Wave Observatory (LIGO) was devised and dedicated to observing such Gravitational-Waves signals. Since then, the detectors have advanced and have an enhanced sensitivity to a wide range of Gravitational-Waves signals as LIGO has successfully detected all three sub-classes of ”compact binaries”: Binary Black Hole (BBH), Binary Neutron Stars (BNS), and Neutron Star-Black Hole Binary (NSBH). Figure 3 depicts the masses of all the Gravitational-Waves detections so far.
The multitude of detections allows us to infer details like the mass, the spin distribution of the NS and BH populations, and their distance from the Earth. Moreover, it has created a great prospect of probing fundamental physics, which comprises comprehending the nature of black holes, constraining cosmological parameters, learning about dark matter and primordial black holes, as well as conducting tests of General Relativity. Moreover, Gravitational-Waves interact very weakly with matter, unlike Electromagnetic radiation.
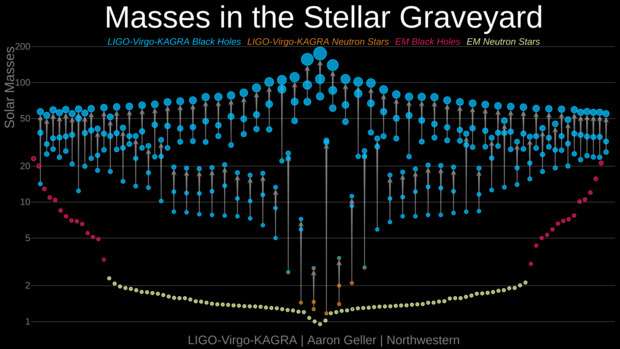
Figure 3: Graphic of masses of announced gravitational-wave detections and black holes and neutron stars previously constrained through electromagnetic observations Credit: LIGO-Virgo / Aaron Geller / Northwestern University.
Therefore, the information carried by Gravitational-Waves about their origins is nearly unhampered, thus providing a holistic approach to view our Universe clearly and comprehend its past, present, and future.
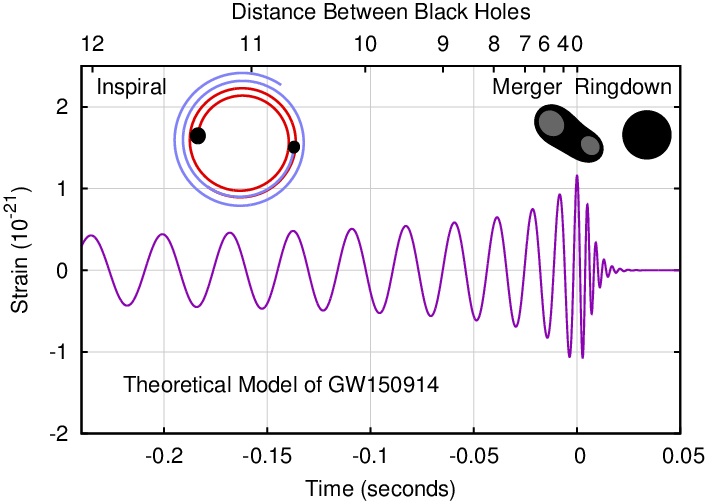
Figure 4: Top. Schematic of Phases of a black hole binary coalescence; Bottom. Typical gravitational wave signal for a black hole binary coalescence.
Credit:http://ccrg.rit.edu/GW150914
What do the Waves tell us?
Albert Einstein predicted the existence of Gravitational-Waves in his General Theory of Relativity; hence, after detecting Gravitational-Waves, the tests of General Relativity (GR) began as a quest to see to what extent this theory is valid. As shown in figure 4, Gravitational-Waves from a compact binary coalescence carry the information of all the three stages of a compact binary merger: the Inspiral, the Merger, and the Ringdown. Therefore, the highly dynamical and radiative space-time associated with the late inspiral, merger, and ringdown of compact binaries enables tests of General Relativity. Accurate waveform modeling plays a crucial role in ascertaining the consistency of the Gravitational-Waves signal data with GR.
With the emphasis on enhancing the detector sensitivities, it will be possible to observe myriad Gravitational-Waves sources with larger Signal-to-Noise Ratios . Hence, this allows the conducting of rigorous tests of GR in the future, which can bring forth diverse physics that may go beyond General Relativity.
Furthermore, the detection of GW170817 marked the advent of Multi-Messenger Astronomy by observing Gravitational-Waves and Electromagnetic Signals from the same source- a merger of a Neutron Star Binary, as depicted in figure 5. This event has provided an opportunity to accurately constrain cosmological parameters like the Hubble constant H0, which is the measure of the local expansion rate of the Universe.
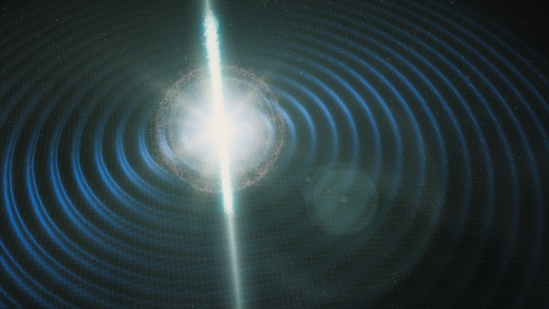
Figure 5: Two neutron stars collide, sending out Gravitational-Waves and electromagnetic radiation detected on Earth in 2017. Credit: FERMILAB
The Gravitational-Waves signal from compact binary systems like binaries of neutron stars or black holes comprises information about the distance to the source and other parameters of the system like the masses of the compact objects. As the Gravitational-Waves signals allow a direct measurement of the distance to the source independent of the Cosmic Distance Ladder method, the term ’Standard Sirens’ was coined for such signals (analogous to Standard Candles). The electromagnetic counterpart to the Gravitational-Waves event allows for the estimation of the red shift of the event. Combining the source distance and the red shift will enable us to probe the cosmic expansion of the Universe as well as other cosmological parameters (like the cosmological constant, matter density, Hubble constant, spatial curvature, etc.). In the future, we hope to constrain the parameters using the dark sirens, which are mergers of stellar-mass binary black holes (SBBHs), and they usually do not have electromagnetic counterparts. In this scenario, in addition to the luminosity distances obtained from Gravitational-Waves signals, the red shift information of sources can be obtained by matching Gravitational-Waves sky localization with galaxy catalogs, allowing the estimation of the Hubble parameter. Therefore in future, with significantly more bright and dark sirens, it will be possible to make robust measurements of H0 and other cosmological parameters.
How can LISA help?
LASER in space sounds surreal, but it will soon be a reality! The LASER Interferometer Space Antenna (LISA) is a space-based Gravitational-Wave detector that will open yet another window to observe GWs in the frequency band of 0.1 mHz - 0.1 Hz. Thus, it offers an exciting opportunity to detect signals from sources such as galactic binaries of white dwarfs and neutron stars, stellar-origin black hole binaries, coalescing massive black hole binaries, binaries formed by a massive black hole, and a stellar-mass compact object known as extreme-mass-ratio inspirals (EMRIs), and a stochastic background generated by cosmological sources. The absence of seismic noise in space and reaching a lower frequency range will allow us to obtain astrophysical information that is very difficult to acquire using ground-based detectors.
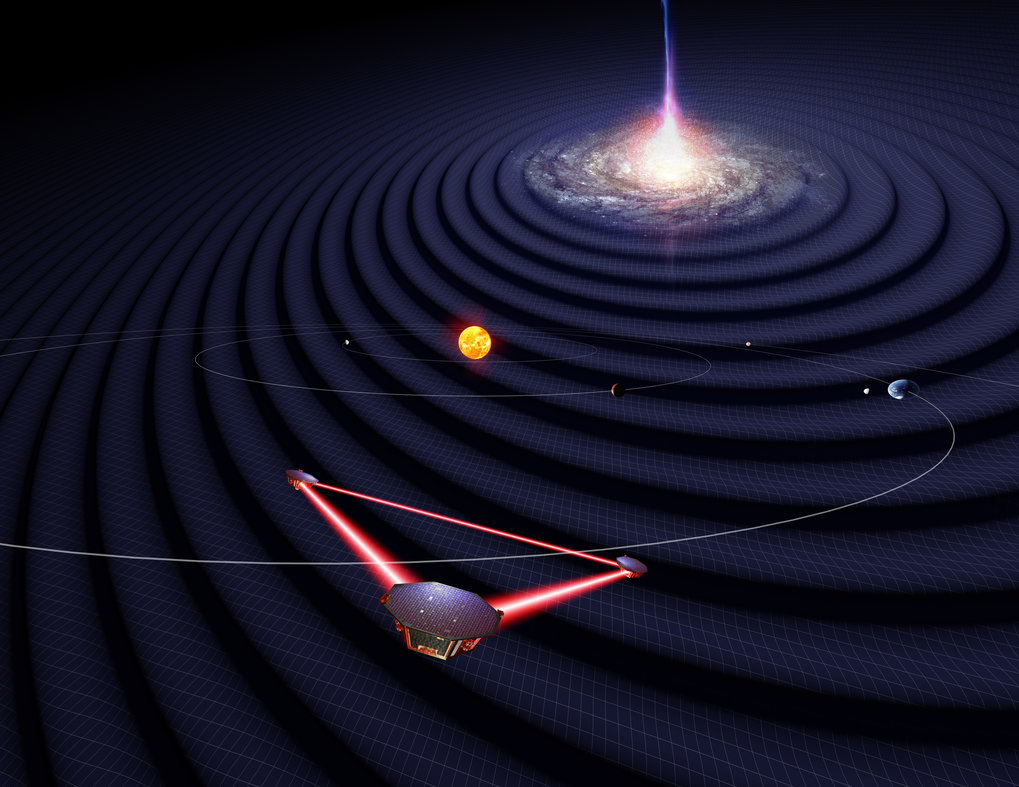
Figure 6: Artist’s impression of the LISA mission. Credits: University of Florida/Simon Barke
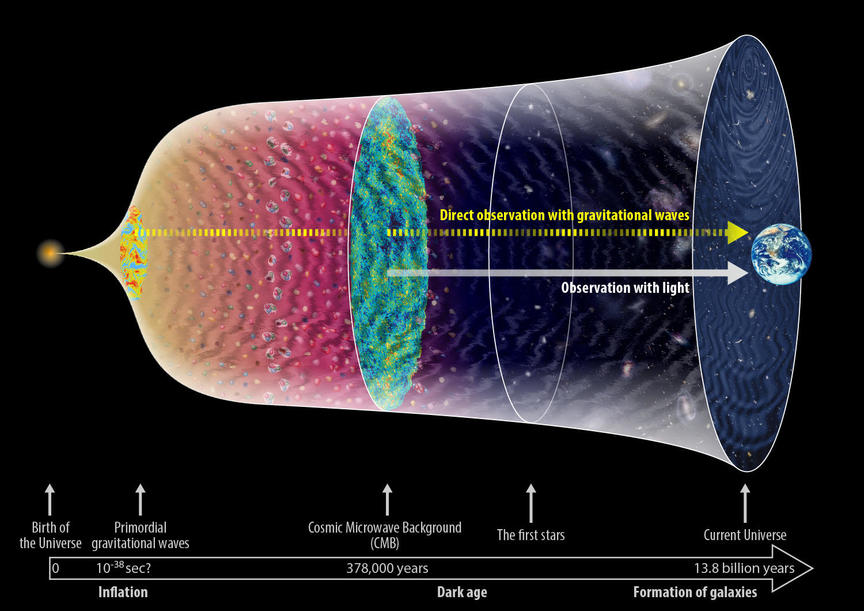
Figure 7: Background Gravitational-Waves from Early Universe. Credits: gwpo.nao.ac.jp
Similar to the Cosmic Microwave Background, a stochastic Gravitational-Wave background as shown in figure 7, might have been formed in the early Universe as a consequence of a large number of spontaneous, independent events. With LISA operating in the low frequency range, creates a possibility for the detection of the stochastic background. This will have a profound impact on our understanding of the physics of the early Universe, and allow us to get a glimpse into the time prior to recombination, which is opaque to electromagnetic Waves. In addition, the ambiguity around the existence of a Dark Massive Object (DMO) in the Galactic centre can be cleared by employing LISA observations of Gravitational-Waves emitted from extreme-mass-ratio inspirals.
An imprint of the space-time surrounding the DMO is encoded in the Gravitational-Waves signal from the host, and we hope that we will be able to extract the required information from the Gravitational-Waves signal allowing us to probe the nature of the DMO.
We are in an exciting phase of Gravitational-Wave astronomy as the diverse possibilities pose an exciting challenge to our existing knowledge of the Universe. This article covers just the tip of the iceberg, as there are more aspects of Gravitational-Waves beyond this article’s scope. The future observing runs of aLIGO and the addition of new detectors like LISA and the Einstein Telescope will create significant avenues to explore the physics of Gravitational-Waves. Also, the evolution of Gravitational-Waves data analysis methods will bolster the task of extracting data from the observed Gravitational-Waves signals. To conclude, one can say that the cosmos has been sending us messages that we have just learned how to receive, and it is a long way till we comprehend these messages entirely.
Glossary
- Observational Run - The gravitational-wave observing schedule is divided into Observing Runs of operation at a given sensitivity of roughly a year duration.
- General Relativity - A theory of gravitation developed by Albert Einstein which says that the observed gravitational effect between masses results from their warping of space-time.
- Space-Time - According to the Theory of General Relativity, the concepts of time and three dimensional space are combined into a four-dimensional space-time continuum.
- Neutron Stars - A compact star that is the collapsed core of a massive super-giant star
- Black Holes - A region of space-time where gravity is so strong that nothing – no particles or even electromagnetic radiation such as light – can escape from it.
- Detector Sensitivity - It is the ability to sense Gravitational Wave Signal. Improving the sensitivity enable interferometers to sense gravitational waves that are weaker and from sources further away and thus reveal important insights into astrophysical events.
- Spin Distribution - Range of rotation speeds in a population of compact stars.
- Inspiral - The first stage of a merger of compact stars (neutron stars or black holes) in binary, where the orbit shrinks due to the emission of gravitational waves.
- Merger - The stage in a merger after inspiral, in which the two compact stars in the binary meet. Gravitational wave emission peaks at this time.
- Ringdown - Immediately following the merger, the now single compact remnant will “ring”. This ringing is damped slowly by the emission of gravitational waves.
- Signal-to-Noise Ratio - It is a measure of the strength of the desired signal relative to background noise (undesired signal).
- Hubble Constant - Expresses the rate at which the universe is expanding. It is a constant of proportionality in the relation between the velocities of remote galaxies and their distances.
- Red shift - The displacement of spectral lines towards longer wavelengths (the red end of the spectrum) in radiation from distant galaxies and celestial objects.
- Multi-messenger Astronomy - Astronomy based on the coordinated observation of different ”messenger” signals like electromagnetic signals, gravitational wave signals and cosmic rays.
- Standard Sirens - A gravitational wave source of known loudness, which provides an independent way of getting at cosmic distances.
- Cosmic Distance Ladder - A step-by-step measurement of the distances to celestial objects.
- Standard Candles - A standard candle is a class of astrophysical objects, such as supernovae or variable stars, which have known luminosity due to some characteristic quality possessed by the entire class of objects.
- Electromagnetic Counterpart - Electromagnetic Waves accompanying Gravitational Waves.
- Spatial Curvature - Describes how space-time is curved and bent by mass and energy.
- Sky localization - The area of the sky that with 90% certainty contains the source location.
- Frequency band of detection - The frequency range of GW signals the detectors are sensitive to and can detect.
- White Dwarfs - White dwarfs are stars that have burned up all of the hydrogen they once used as nuclear fuel. They are very dense: their masses are comparable to that of the Sun, while their volume is comparable to that of Earth.
- Stochastic Background - A random gravitational-wave signal from different sources. The sources of the stochastic background can be broadly divided into two categories: cosmological sources, and astrophysical sources.
- Cosmological Sources - Cosmological gravitational-wave backgrounds produced by sources that existed in the early Universe just a few seconds after the Big Bang.
- Cosmic Microwave Background - Relic electromagnetic radiation from the early universe. It is an important source of data on the early universe because it is the oldest electromagnetic radiation in the universe.
- Recombination - In cosmology, recombination refers to the epoch after the Big Bang when the universe was sufficiently cool for electrons and nuclei to form atoms for the first time, and the universe became transparent to radiation.
- Dark Massive Object - A high mass object or cluster of objects at the centre of a large stellar system, such as a galaxy or globular cluster.
- Waveform Models - These are used in gravitational wave data analysis to detect and then to measure the properties of a source by matching the model waveforms to the signal from a detector.
Further Reading
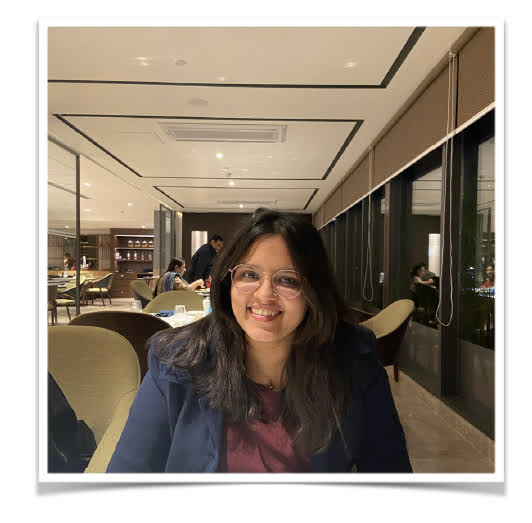
Gopi K. Patel, is an incoming Ph.D. student at the Institute of Cosmology and Gravitation, University of Portsmouth. She will be working on the prospects of GW science with LISA and LIGO. She also likes to paint, play violin and is a plants-person.